Followers | 206 |
Posts | 18506 |
Boards Moderated | 0 |
Alias Born | 10/30/2016 |
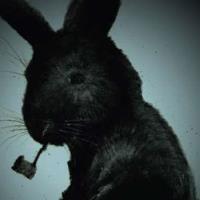
Wednesday, June 21, 2017 10:11:59 PM
Abstract:
Interest in electromagnetic field (EMF) treatments has increased rapidly in recent years due to its advantages over other treatments for tissue healing and infection. Benefits include low-cost, ready availability, ease of localized application, few if any side-effects, and indefinite shelf life. Immunological studies show that low-intensity EMF can interact with cells and tissues, providing a large number of anti-inflammatory and wound healing applications. The effect of EMF on the immune system in phagocytic cells alone has attracted attention because of the role that extremely low-frequency electromagnetic field (ELF-EMF) plays in decreasing the growth rate of bacteria. With today’s antibiotic-resistant bacteria, medicine is in need of a mechanism to aid in the control of inflammatory response, greatly benefitting the fields of disease pathology, tissue engineering and regenerative medicine.
Keywords:Electromagnetic field (EMF), Immune response, Energy medicine, Inflammation
1. A brief history of energy medicine and electromagnetic field therapy
The earliest recorded use of energy medicine (EM) dates back to 2750 BC, when patients were exposed to shocks produced by electric eels in order to heal different maladies [1]. The next recorded use was around 400 BC, when a Greek philosopher named Thales of Miletus rubbed amber and obtained static electricity believed to cure afflictions [2]. In 1929, Yale University anatomy professor Harold Saxton Burr began studying the role of electricity in disease. In his book Blueprint for Immortality: the Electric Patterns of Life, he explains how he was able to measure human energy fields with standard detectors. Modern research has confirmed the observations of Burr by concluding that both healthy and unhealthy people produce electrical charges and changes in magnetic fields (MFs) around their body [3]. In the 1960s electrical engineers Gerhard Baule and Richard McFee used a pair of run coils to detect a magnetic field (MF) produced by the electrical activity of heart muscle [4]. Shortly afterward Willem Einthoven discovered that heart electricity could be recorded with a galvanometer, and electrocardiograms (EKGs) and began using it as a standard tool for medical diagnosis. Shortly after Einthoven received the Nobel Prize for his discovery of heart electricity, Hans Berger showed that much smaller electrical fields could be recorded coming from the brain using electrodes attached to the scalp. This device is used to categorize health and disease of the brain and is known as the electroencephalograph (EEG) [5]. Today EEGs record electrical activity along the scalp and measure voltage fluctuations resulting from ionic current flows in neuronal brain cells [6]. In the 1970s and 80s, orthopedic surgeon Robert O. Becker conducted research in electrophysiology when he observed that properties of connective tissue (cells, fibers, and extracellular matrix) surrounding the nervous system were operating on a direct current [7, 8]. Like Burr, Becker thought some sort of field surrounded the body, stimulating regeneration. He discovered that an electrostatic field could enable regeneration of a frog limb [8]. He also reported that direct current (about 1 nanoampere) through a broken bone radically improves the growth and repair of bones [9]. Becker showed the part of the connective tissue layer called the perineurium (sheath surrounding nerve fibers) sets up a low-voltage current that controls injury repair. One of Becker’s most important discoveries is that the perineurium is sensitive to energy fields [10, 11]. Once it was discovered the body produces electrical and MFs, it was then comprehensible how EMF devices could measure and modulate them. Becker’s work has been substantiated by current research from Hannemann et al. [12] and Saliev et al. [13].
Pulsed electromagnetic field (PEMF) therapy has been FDA-approved to fuse bones and reduce swelling and joint pain [14]. PEMF has been used to treat pain and edema in soft tissue for over sixty years [15]. It has been firmly established that tissues such as blood, muscle, ligaments, bone and cartilage respond to biophysical input including electrical and electromagnetic fields (EMFs) [12, 15-20]. Research shows that certain field strengths and frequencies of PEMF appear to be disease modifying. For example, treatments of 10-25 Hz frequency decreased pain and improved range of motion for osteoarthritis patients [21], improvement of cognitive development reversal of brain amyloid-ß (Aß) deposition in Alzheimer’s patients using 25-50 transcranial electromagnetic field treatment (TEMT) [22], improved function, pain and fatigue in fibromyalgia patients using 25-50 Hz field [23], and a 27.12MHz field tuned to tissues specific field strengths has been shown to block tumor growth in cancer patients [24]. PEMF devices can be attuned to certain vibrational frequencies and field strengths in order to stimulate hormones [25], growth factors [26] and interleukins [27]. PEMF has also been reported to reduce pain and inflammation after traumatic brain injury [28], decrease osteoarthritic inflammation [18], and reduce neuropathic pain [29], as well as control the growth of lymphocytes [30].
2. How is an EMF generated?
Faraday’s Law of Induction and Maxwell’s equations explain how electromagnetic field (EMF) is generated. A static electric field (EF) is generated by a static charged particle. The EF (or E component of an EMF) exists whenever charge is present. Its strength is measured in volts per meter [V/m]. An EF of 1 V/m is represented by a potential difference of 1 V existing between two points that are 1 m apart. The V/m is primarily used to express the intensity of the EMF. MF (or M component of an EM field) arises from current flow. Its flux density is measured in tesla (T) in the International System (SI), and in gauss (G) which is the former CGS system of units (1 G = 10-4 T or 1 T = 10 milligauss). A flux density of 1 G represents 1 Maxwell / cm2. The tesla (or Gauss) is mainly used to denote the flux density (intensity) produced by MFs. Both EFs and MFs are generated if a charged particle moves at a constant velocity. EMFs are generated when a charged particle is accelerated. Most often this acceleration takes place in the form of an oscillation, therefore electric and MFs often oscillate. Change in the EF creates a MF, and any change in the MF creates an EF. This interaction suggests the higher the frequency of oscillation, the more the electric and MFs are mutually coupled. EMF devices include solenoids, transcutaneous electrical nerve stimulation (TENS), and Helmholtz coils.
3. EMFs effect on biosystems
The biological effects of low-frequency EMF have been the subject of numerous studies since they can penetrate deep into tissues [31-38]. It has been shown that low-frequency EMF can act at the cellular level affecting various cell functions, including cell proliferation and differentiation [39-42], apoptosis [43-45], DNA synthesis [46, 47], RNA transcription [48], protein expression [49], protein phosphorylation [50], re-dox mediated rises in the inflammatory transcription nuclear factor kappa B (NFkB) [51, 52], microvesicle motility [53], ATP synthesis [54], hormone production [55], antioxidant enzyme activity [56], metabolic activity [57], and the inhibition of adherence [58]. The regulation of binding activity and signal amplification to a biological response has traditionally been thought to be driven solely by chemical messengers and receptors, but now research shows that molecular mechanisms may not be the only factors involved in biological communication. Chemical messengers are perhaps the particle components modulated by governing energy fields underlying signal transduction. This theory would answer such critical questions as: 1)What coordinates biological processes? 2) What directs the correct number of molecules at specific times to perform specific functions? 3) How does coupling to the receptor trigger the response? Cells are able to recognize each other and their surroundings in order to find their proper destinations in an organism, even when the conventional routes are blocked [59, 60]. Cells of the same type tend to aggregate and actively preserve this activation in situ and in vitro. There are indications that the speed of the EMF signal clearly exceeds the speed range of molecular transmission [61]. Electrical properties such as membrane surface charge and potential are especially influenced by ELF-EMF [62-64]. EMFs are usually not represented in the processes taking place in living cells which is dominated by microscopic morphology, biochemistry and molecular genetics. The biochemical model of cell communication and signal transduction clearly needs a biophysical component.
Most of the models explaining how weak EMFs interact with biological systems suggest a sub-cellular organelle or single molecule as the target [65-72]. Fritz-Albert Popp was one of the first pioneers to research delocalized cellular EMFs and their interference [73]. Based on his theories every biological system displays a complex wave pattern that is most likely tissue specific. Disruption of these complex patterns can occur through interference of both inter- and intra-cellular communication [74]. If cells can communicate with each other through fields, then it is possible that an EMF can alter the information being exchanged [75]. Studies in developmental biology have identified key regulators of developmental properties, and have indicated where endogenous EMF are located in the action potentials of nerves and heart tissue, and in skeletal muscle vibrations, with frequencies elicited by rhythmic activities throughout the human organism [76]. Endogenous EMF frequencies are normally in the range of extremely-low (5-60 Hz) [77]. It is these endogenous frequencies which can be entrained to follow exogenous EMF of the same frequencies. This entrainment (via harmonic resonance) is what influences the modulatory effects of EMF on cells and tissues. While there are a multitude of research articles investigating this phenomena, the methods for gathering these data include an overwhelming array of experimental models, EMF devices, waveforms, and clinical applications; therefore a consensus of standardized methods for experimentation is greatly needed to determine which responses directly result from the EMF exposure. Effective EMF stimuli are coherent, presenting a series of recurring signals that must be present for a minimum amount of time [78]. Therefore, the precise time points during which differentiation occurs under EMF stimuli need to be elucidated. Uncertainty still exists as to the mechanisms by which extra-low frequency and high- frequency levels of EMF act at the cell and molecular level. While high frequency (900-1800 MHz) EMF such as that derived from microwave and mobile phone communication, act through mixtures of modulated and carrier frequencies, research to-date has focused primarily on the thermal effects of radiation at a tissue-specific absorption rate known as SAR.
4. How does EMF therapy work?
The mechanism for action of EMF on cells and tissues is based on how cells can both generate EMF endogenously and detect EMF exogenously. Biological systems such as cells communicate not only with each other but also interact with their environment [79, 80]. This is done through several mechanisms at many levels depending on the type of cell tissue and nature of the information being communicated. Most known mechanisms in the literature address cell-cell interaction as chemical or electrical signaling, but intercellular interaction can also be attributed to EMFs [76, 81, 82]. In 1935, Burr et al. published a report on stable voltage gradients in various biological systems [83]. Since then researchers have discovered that these stable gradients can be altered when the whole organism undergoes biological processes such as growth, localized injury, and microbial invasion. Because EMF radiates, it behaves in a wave-like manner. It has been suggested that the initial interaction occurs outside the plasma membrane, but could also involve interactions with transmembrane proteins [84, 85]. For example, NIH3T3 cells exposed to a 50 Hz PEMF for over 2 h significantly increased the clustering of intermembrane proteins compared with controls [86]. Investigators concluded that the signal was likely being propagated and amplified through intracellular signal transduction pathways [87]. An example of this is when the calcium stored in the intracellular compartment prompting mitochondria to produce free radicals (which increase DNA response) [88] can be controlled by PEMF [89], providing a first order effect in preventing the onset of inflammatory responses. The impact of EMF on calcium channel protein has been reported many times [90-93]. The genes that encode ion channels are important because they produce the gradients that determine downstream cell behavior. Increased understanding of how these mechanisms work will lead to devices that stimulate cell treatment directly to the damaged region producing the bioelectromagnetic changes needed to repair and regenerate tissues.
5. EMF and Inflammation
EMF has many well documented physiological effects on anti-inflammatory mechanisms. EMF therapies can provide noninvasive, safe and easy to apply methods to directly treat the source of pain, injury, inflammation and dysfunction [94, 95]. Low-frequency EMF has a long record of safety, backed by tissue culture, animal and clinical studies which have been conducted for over four decades [96]. Although the exact mechanism of action is unclear, the cell membrane is most often considered the main target for EMF signals [94]. It has been reported that EMF affects membrane mediated signal transduction processes, especially the Ca2+ transport system [97-99]. Early events in signal transduction play a critical role in calcium influx in the lymphocyte. Because calcium is an important second messenger for a wide variety of important cellular processes such as RNA, DNA and protein synthesis; modulation of calcium signaling by EMFs has the potential to influence these cell functions [100]. Studies have demonstrated that EMF can stabilize the cell membrane by restoring membrane protein activity (Ca2+ -ATPase) and maintain intracellular Ca2+ levels [101, 102]. Biological systems in transition have been shown to be more sensitive to EMF exposure than in stationary systems. In one study immune compromised animals constituting systems in transition state were shown to be more sensitive to EMF exposure; whereas healthy animals, considered to be in relatively stable systems, exhibiting no sensitivity to the same field parameters [103]. Low-frequency, low-intensity EMF can be beneficial in reducing inflammation without potential side effects indicating its value as a viable alternative for treating inflammatory responses.
Inflammation involves the activation of the immune system in response to infection, irritation or injury. It is characterized by an influx of white blood cells, redness, swelling, heat, pain, and the dysfunction of organs involved. Inflammation is the first response to healing wounds and fighting infection. Without an acute inflammatory response the body would not be able to repair itself and regenerate tissue; however when the immune system is always activated, acute inflammation becomes chronic, which can lead to chronic disease states [104]. Inflammation plays a key role in the development of diseases such as arthritis [105], diabetes [106], heart disease [107], Alzheimer’s disease [108], obesity [109], irritable bowel syndrome[110], Parkinson’s disease [111], cancer [112], HIV/AIDS [113] and every type of autoimmune disease [114]. Disorders in which inflammation plays an important pathogenetic role include: anaphylaxis, Ankylosing spondylitis (spinal arthritis), asthma, atherosclerosis, atopic dermatitis, chronic obstructive pulmonary disease, Crohn’s disease, gout, Hashimoto’s thyroiditis, Ischaemia-reperfusion injury, multiple sclerosis, osteoarthritis, pemphigus (skin blistering), periodic fever syndromes, psoriasis, rheumatoid arthritis, sarcoidosis (chronic lung disease), systemic lupus erythematosus, Type 1 diabetes melilitus, ulcerative colitis, and xenograft rejection [115]. Inflammation can contribute as much to pathology as microbial toxicity in diseases such as cystic fibrosis pneumonitis, Hepatitis C, influenza virus pneumonia, leprosy, sepsis and tuberculosis.
For decades researchers have realized that inflammation is the same in different diseases, and a better understanding of inflammation may lead to better treatments for numerous diseases. Neurologists have studied the effect of inflammation on brain injury and irritation discovering that glial cells emit chemical signals known as cytokines which begin the inflammatory process [116]. In diseases like Alzheimer’s, these glial cells are too highly activated, creating a chronic inflammatory response in the brain. Inflammation also plays a role in heart disease because the immune system attacks low-density lipoprotein (LDL) cholesterol embedded in arterial walls. Chronic inflammation will damage arteries causing them to burst [117]. Inflammation has also been linked to diabetes [114]. In type-1 diabetes, the immune system attacks beta cells that make insulin. There is also strong evidence that chronic inflammation can promote cancer growth [118]. While acute inflammation is necessary for normal development of the immune system and the organs involved, chronic inflammation can be detrimental to the entire organism. To be able to treat this condition with a non-invasive, low-cost, easy-to-use complement or alternative to currently prescribed treatments, without the debilitating side-effects of pharmaceuticals and surgery would advance the field of pathology, and also improve regenerative medicine techniques during surgery and after implantation.
6. Mechanisms initializing inflammatory response
Mechanisms initializing inflammation focus on the recruitment of leukocytes in the blood [119]. Rapid response requires sentinel cells, such as mast cells (first responders) and macrophages (phagocytes), to position themselves at the site of injury. Perivascular mast cells release histamine, eicosanoids, pre-formed tumor necrosis factor (TNF), newly synthesized cytokines, tryptases, proteases, and chemokines. Histamine, eicosanoids and tryptases cause vasodilation (responsible for the heat and redness) and extravasation of fluid (causing swelling) [115]. TNF partially activates the neutrophils (along with mast cells) as well as chemokines and cytokines. Oxidants activate matrix metalloproteinases (MMPs) and inactivate protease inhibitors causing tissue breakdown [120]. MMPs cleave TNF from tissue macrophages as well as monocytes which are attracted from the bloodstream, through the vessels, and into the tissue by azurocidin, a cationic anti-microbial protein [121]. Activated macrophages secrete proteases, eicosanoids, cytokines, reactive oxygen species (ROS), and reactive nitrogen intermediates (RNIs). The inflammatory system is designed for lag-free acceleration, but requires on-going verification of an emergency to avoid defaulting to the resting state [115]. Each newly recruited cell releases pro-inflammatory signals after integrating the origin of both the host and microbe. As long as the host and microbial pro-inflammatory stimuli predominate, macrophage derived chemokines continue to attract neutrophils; and reactive oxygen intermediator (ROI) and hyaluronidase from macrophages and neutrophils break down hyaluronic acid in the extracellular matrix into low molecular weight fragments. Macrophages then ingest apoptotic neutrophils and degrade residual elastase. Macrophages and T-lymphocytes work together to produce various cytokines that have both pro- and anti-inflammatory effects [122]. Pro-inflammatory cytokines such as interleukins promote chronic vascular inflammation. Interleukin 1 (IL-1) is a prototypic pro-inflammatory cytokine that expresses as both IL-1a and IL-1ß, which are mediated through the IL-1 receptor (IL-1R). After binding to IL-1R, IL-1 produces a broad spectrum of cytokines and chemokines, as well as producing adhesion molecules on endothelial cells (EC), which recruit inflammatory cells [123]. Interleukin-6 (IL-6) is a multifunctional cytokine that regulates various aspects of the immune response, acute-phase reaction, and haematopoiesis [124]. IL-10 is an anti-inflammatory cytokine that plays an active part in the adaptive immune responses. Dysregulation of IL-10 is linked to numerous infectious and autoimmune diseases [125]. IL-10 contributes to the delicate balance between inflammation and immunoregulation. Members of the TNF and TNF-receptor super family are instrumentally involved in the maintenance of homeostasis in the immune system [126]. TNF not only provides beneficial and protective effects in inflammation and host defense, it can also be responsible for damaging effects in sepsis, cachexia, and autoimmune diseases.
Understanding the role of inflammation in disease involves studying the activation of endogenous molecules in response to injury or infection. Promoting an inflammatory response involves focusing on a combination of signals that mimic injury of the host and the presence of infectious agents. Anti-inflammatory therapies must interrupt signals that cause acute phase inflammatory responses to become chronic. Unfortunately the redundancy of multiple signals complicates this goal; but the recognition of down regulatory signals offers opportunities to stop inflammation before it wreaks havoc on the immune system. However there is still the dilemma that the more extensively a therapy suppresses inflammation, the more likely it will exacerbate infection. Experimental therapies are uncovering a wealth of biomolecular detail as systems biology is beginning to understand the complexity and dynamics of the inflammatory process. A collaboration between experimental and systems biology would be a powerful alliance to identifying points of control amendable to relatively safe and effective interventions.
7. Model for studying the effect of EMF on inflammation
Acute or chronic inflammation is a multi-step process mediated by immune cells, of which macrophages play a central role in regulating many different immunopathological aspects of the inflammatory response. Immunological cellular events are managed by surface receptors such as toll-like receptors (TLR-4 for example) [127]. Activation of these molecules by inflammatory stimuli such as lipopolysaccharide (LPS) in macrophages can upregulate intracellular signaling and gene expression through the activation of transcription factors such as the nuclear factor kappa light-chain-enhancer B(NFkB) [128]. Primary macrophages and cancerous macrophage-like cells (e.g. RAW 264.7 cell lines) induced by LPS are now regarded as a useful tool for evaluating anti-inflammatory mechanisms and the potency of anti-inflammatory drugs, due to their ability to display similar inflammatory responses [129]. LPS is a component of the outer membrane of the cell wall of Gram-negative bacteria. It is the main component of Gram-negative bacteria that activates the host immune system and is crucial in fighting pathogens as well as in the induction of sepsis. Macrophages are cells of the innate immune system that react rapidly to a variety of microbes. To shorten their reaction time, they store messenger ribonucleic acid (mRNA), a molecule of RNA that encodes a chemical “blueprint” for a protein product of proinflammatory cytokines, in their cytoplasm. Macrophages release pre-synthesized tumor necrosis factor (TNF) from secretory granules immediately in response to bacteria [130]. Macrophages ingest bacteria via phagocytosis, destroying them within their phagolysosomes, and present the components of the bacteria to T-cells. TNF release from the secretory granules occurs in sync with phagocytosis of the microbes. This happens rapidly and does not require fresh mRNA and protein synthesis. TNF is detectable within 15 minutes of LPS exposure in the supernatant of stimulated immune cells [131]. Both bacteria and LPS trigger the NFkB pathway in macrophages. In order to simulate this inflammatory reaction LPS was used to initiate an inflammatory response in RAW 264.7 murine macrophages. Different dosage levels and time points were tested to determine best outcomes. LPS dosage was optimized at 3 h after induction to 8µl/ml using NFkB assay. Newly synthesized TNF also appeared in the supernatant of macrophages later on, which was tested using TNF enzyme-linked immunosorbent assay (ELISA) for murine model. LPS activation of TLR-4 is mainly responsible for the activation of the NFkB pathway when macrophages are exposed to Gram-negative bacteria. NFkB is a protein complex that controls the transcription of DNA. NFkB is found in almost all animal cell types and is involved in cellular responses to stimuli such as stress, cytokines, free-radicals, ultraviolet radiation, oxidized LDL, and bacterial or viral antigens [132]. NFkB is used by cells to regulate genes that control cell proliferation and cell survival. Active NFkB turns on the expression of genes that keep the cell proliferating and protect it from situations that would cause it to die through apoptosis. Defects in NFkB result in increased susceptibility to apoptosis which leads to increased cell death. This is because NFkB regulates anti-apoptotic genes and checks the activity of caspase enzymes which are central to apoptotic processes [133]. NFkB contributes to the induction of four classes of genes: 1) genes that have products involved in the negative-feedback control of NFkB activity; 2) genes that have products that serve various immunoregulatory functions; 3) genes that have products that inhibit caspase activation and apoptosis; and 4) genes that promote cell proliferation (see Figure 1).
NFkB activation leads to the transcription of genes of inducible NO-synthase, and pro-inflammatory cytokines such as TNF-a, IL-1b, IL-6, and IL-10.
Fig. 1 Shows how NFkB contributes to the induction of four classes of genes.
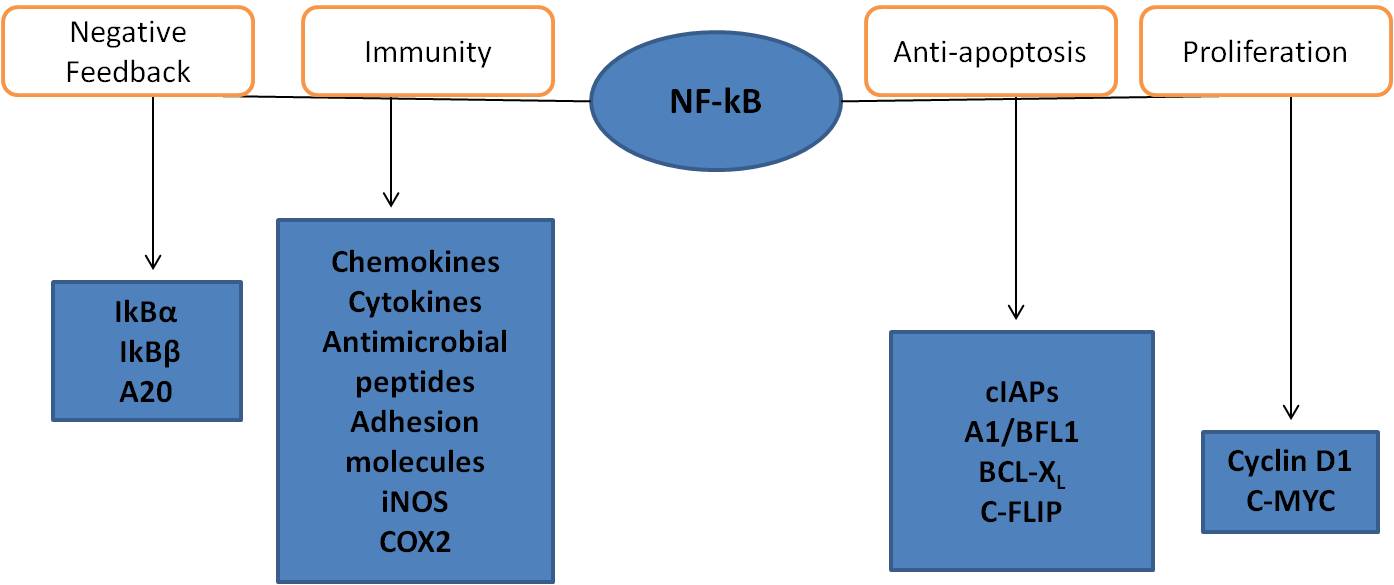
8. Effect of EMF on cytokine production
It is now well established that exogenous applied EMF affects cell signaling and cytokine production. In a model studying osteoarthritis, EMF showed statistically significant improvement in stimulating transforming growth factor beta (TGF-ß) [134]. Since TGF-ß is understood to upregulate gene expression for aggrecan (a cartilage-specific core protein), downregulate matrix metalloprotease (MMP) and IL-1 activity, and upregulate inhibitors of MMP, the stimulation of TGF-ß could be considered a mechanism by which EMF favorably affects cartilage homeostasis.
Application of EMF does not appear to alter the cell immunophenotype of fibroblast-like cell populations, but does appear to decrease the production of inflammatory cytokines IL-1ß and TNF-a. EMF also appears to increase anti-inflammatory cytokine IL-10 [135]. Both IL-1 and TNF-a concentration in the synovial fluid were significantly lowered while TGF-ß was significantly higher compared with controls. Large bone formation was also observed one month after osteochondral graft implantation using EMF treated grafts which favor early graft stabilization [136]. EMF exposure at 75 Hz, 45 mT limited bone resorption in the subchondral bone while cytokine assessment in the synovial fluid indicated a more favorable articular environment for the graft.
9. Effect of EMF on NFkB
Effect of EMF on NFkB has been shown to be both promising as an inflammatory inhibitor and as a minimal effecter of downstream effects on gene expression. Human keratinocytes exposed to 50 Hz at 1 mT for different lengths of time and compared with unexposed control cell [137]. Cell growth and viability were evaluated along with chemokine production and expression and real-time polymerase chain reaction (RT-PCR). Significantly increased growth rates were observed in cells after 48 h of EMF exposure as compared with control. NFkB levels became almost undetectable after only 1 h of EMF exposure, and were inversely correlated with cell density. Results showed that extra-low frequency electromagnetic field (ELF-EMF) modulates chemokine production and keratinocyte growth through the inhibition of NFkB signaling pathways thereby inhibiting inflammatory processes. ELF-EMF as low as 5 Hz and 1.5 mT has been shown to modulate chemokine production and keratinocyte growth through inhibition of the NFkB signaling pathway, thereby decreasing inflammatory processes [138, 139]. Natarajan et al. investigated whether monocytes exposed to a PEMF of 1 kV/cm triggers a signaling pathway responsible for the transcriptional regulation of NFkB-dependent gene expression [140]. The authors reported that PEMF exposure did not induce NFkB-driven luciferase activity in these cells, indicating that the activation of NFkB at 24 h after the EMF exposure is functionally inactive. From these results, they postulated that the EMF-induced NFkB activation was only a transient response, with no minimal or no downstream effect; however, they did not take into consideration that long term molecular effects could be frequency dependent, and this particular frequency only effected the cellular response in a limiting manner.
10. Conclusion
The inflammatory response is a well-organized system of signals that control and maintain homeostasis in the immune system. An imbalance in the up- or down-regulation of these signals can leave inflammation unchecked, resulting in cell and tissue damage. EMF has been shown to be clinically beneficial in the repair of many types of cells and tissues through membrane and cytoskeletal organization in receptor and kinase activity [141-144]. Because the cell membrane has been proposed as the primary site of transduction for EMF effects, relevant mechanisms of action may include changes in cell membrane binding and transport processes, displacement or deformation of polarized molecules, and modifications in the conformation of biological water [145, 146]. Although it is not well understood how an extremely low-frequency MF affects cytokine production, studies have shown that the outcome of EMF interactions significantly depends on the metabolic states of the cells during exposure [147-150]. Cells in a metabolically stable state can counteract weak cellular changes so that no significant biological alterations occur, but when homeostatic changes such as inflammation overwhelm a regulatory system, the organism will move into an imbalanced state. Cells in unstable homeostatic states, where they have been invaded with stressors like traumatic injury or pathogens, are more susceptible to EMF resonances because of their instability. This theory would account for the lack of effect of EMF on healthy cells, such as in Zimmerman’s work with EMF on tumor growth [151]. Homeostasis in the immune system is dependent on a balance between responses that control infection and tumors, and the reciprocal responses which prevent inflammation and autoimmune diseases [152]. It is possible that an EMF is able to affect cytokine production due to the imbalance caused by inflammatory response in cells and tissues. To be able to use this mechanism to control inflammatory response of disease, and during regenerated tissue and organ transplantation, would greatly benefit the fields of disease pathology, tissue engineering and regenerative medicine.
References
[1] Kellaway P. The part played by electric fish in the early history of bioelectricity and electrotherapy. Bulletin of the History of Medicine. 1946;20.
[2] Babbitt E. The principles of light and color. College of Fine Forces. 1873;East Orange, NJ.
[3] Oschman J. Energy Medicine: The Scientific Basis. Churchill Livingstone. 2000;Edinburgh.
[4] Baule G, McFee R. Detection of the magnetic field of the heart. Amer Heart Journal. 1963;66.
[5] Berger H. Uber das elektrenkephalogramm des meschen. Archiv fur Psykchiatria. 1929;87.
[6] Niedermeyer E, da Silva F. Electroencephalography: Basic Principles, Clinical Applications, and Related Fields Lippincot Williams & Wilkins 2004. Baltimore, MD.
[7] Becker R. The bioelectric factors in amphibian-limb regeneration. J Bone Joint Surg Am. 1961;43:643.
[8] Becker R, Spadaro, JA. Electrical stimulation of partial limb regeneration in mammals. Bull N Y Acad Med. 1972;48:627.
[9] Becker R, Spadero J. Electrical stimulation of partial limb regeneration in mammals. Bull 1972. NY Acad Med:627.
[10] Becker R. The Body Electric: Electromagnetism and the Foundation of Life. New York 1985. William Morrow & Co., Inc.
[11] Becker R. Cross Currents: the Perils of Electropollution, the promise of electro-medicine. Jeremy P Tarcher 1990. Los Angeles, CA.
[12] Hannemann P, Mommers EH, Schots JP, Brink PR, Poeze M. The effects of low-intensity pulsed ultrasound and pulsed electromagnetic fields bone growth stimulation in acute fractures: a systematic review and meta-analysis of randomized controlled trials. Arch Orthop Trauma Surg. 2014;134:1093.
[13] Saliev T, Mustapova Z, Kulsharova G, Bulanin D, Mikhalovsky S. Therapeutic potential of electromagnetic fields for tissue engineering and wound healing. Cell Prolif. 2014;47:485.
[14] Nelson F, Zvirbulis R, Pilla A. The use of a specific pulsed electromagnetic field (PEMF) in treating early knee osteoarthritis Trans 56th Annual Orthopaedic Research Society Meeting 2010. New Orleans, LA:1034.
[15] McKay J, Prato F, Thomas A. A literature review: The effects of magnetic field exposure on blood flow and blood vessels in the microvasculature. Bioelectromagnetics. 2007;28:81.
[16] Vignola M, Dávila S, Cremonezzi D, Simes JC, Palma JA, Campana VR. Evaluation of inflammatory biomarkers associated with oxidative stress and histological assessment of magnetic therapy on experimental myopathy in rats. Electromagn Biol Med. 2012;Jul 19.
[17] Currier D, Ray JM, Nyland J, Rooney JG, Noteboom JT, Kellogg R. Effects of electrical and electromagnetic stimulation after anterior cruciate ligament reconstruction. J Orthop Sports Phys Ther. 1993;17:177.
[18] Fini M, Torricelli P, Giavaresi G, Aldini NN, Cavani F, Setti S, Nicolini A, Carpi A, Giardino R. Effect of pulsed electromagnetic field stimulation on knee cartilage, subchondral and epyphiseal trabecular bone of aged Dunkin Hartley guinea pigs. Biomed Pharmacother. 2008;62:709.
[19] Hannemann P, van Wezenbeek MR, Kolkman KA, Twiss EL, Berghmans CH, Dirven PA, Brink PR, Poeze M. CT scan-evaluated outcome of pulsed electromagnetic fields in the treatment of acute scaphoid fractures: a randomised, multicentre, double-blind, placebo-controlled trial. Bone Joint J 2014;96-B8.
[20] Assiotis A, Sachinis NP, Chalidis BE. Pulsed electromagnetic fields for the treatment of tibial delayed unions and nonunions. A prospective clinical study and review of the literature. J Orthop Surg Res. 2012;7:24.
[21] Li S, Yu B, Zhou D, He C, Zhuo Q, Hulme JM. Electromagnetic fields for treating osteoarthritis. Cochrane Database Syst Rev 2013;12:CD003523. doi: 10.1002/14651858.CD003523.pub2.
[22] Arendash G. Transcranial electromagnetic treatment against Alzheimer's disease: why it has the potential to trump Alzheimer's disease drug development. J Alzheimers Dis. 2012;32:243.
[23] Sutbeyaz S, Sezer N, Koseoglu F, Kibar S. Low-frequency pulsed electromagnetic field therapy in fibromyalgia: a randomized, double-blind, sham-controlled clinical study. Clin J Pain. 2009;25:722.
[24] Zimmerman J, Jimenez H, Pennison MJ, Brezovich I, Morgan, D, Mudry A, Costa FP, Barbault A, Pasche B. Targeted treatment of cancer with radiofrequency electromagnetic fields amplitude-modulated at tumor-specific frequencies. Chin J Cancer. 2013;32:573.
[25] Schnoke M, Midura, R. Pulsed electromagnetic fields rapidly modulate intracellular signaling events in osteoblastic cells: comparison to parathyroid hormone and insulin. J Orthop Res. 2007;25:933.
[26] Chalidis B, Sachinis N, Assiotis A, Maccauro G. Stimulation of bone formation and fracture healing with pulsed electromagnetic fields: biologic responses and clinical implications. Int J Immunopathol Pharmacol. 2011;24:17.
[27] Cossarizza A, Monti D, Bersani F, Paganelli R, Montagnani G, Cadossi R, Cantini M, Franceschi C. Extremely low frequency pulsed electromagnetic fields increase interleukin-2 (IL-2) utilization and IL-2 receptor expression in mitogen-stimulated human lymphocytes from old subjects. FEBS Lett. 1989;248:141.
[28] Rasouli J, Lekhraj R, White NM, Flamm ES, Pilla AA, Strauch B, Casper D. Attenuation of interleukin-1beta by pulsed electromagnetic fields after traumatic brain injury. Neurosci Lett.2012;519:4.
[29] Weintraub M, Cole SP. Pulsed magnetic field therapy in refractory neuropathic pain secondary to peripheral neuropathy: electrodiagnostic parameters--pilot study. Neurorehabil Neural Repair. 2004;18:42.
[30] Jasti A, Wetzel BJ, Aviles H, Vesper DN, Nindl G, Johnson MT. Effect of a wound healing electromagnetic field on inflammatory cytokine gene expression in rats. Biomed Sci Instrum. 2001;37:209.
[31] Marino A, Becker R. Biological effects of extremely low frequency electric and magnetic fields: a review. Physiological Chemistry and Physics. 1977;9:131.
[32] Adey W. Frequency and power windowing in tissue interaction with weak electromagnetic fields. Proceedings of the IEEE. 1980;68:119.
[33] Robinson K. The responses of cells to electrical fields: a review. Journal of Cell Biology, 1985, 101:2023.
[34] Frey A. Electromagnetic field interactions with biological systems. FASB J. 1993;7:272.
[35] Hong F. Magnetic field effects on biomolecules, cells and living organisms. Biosystems. 1995;36:187.
[36] Volpe P. Interactions of zero-frequency and oscillating magnetic fields with biostructures and biosystems. Photochemical and Photobiological Sciences. 2003;2:637.
[37] Funk R, Monsees T. Effects of electromagnetic fields on cells: physiological and therapeutical approaches and moleclular mechanisms of interaction. Cell Tissues Organs. 2006;182:59.
[38] Funk R, Monsees T, Ozkucur N. Electromagnetic effects: from cell biology to medicine. Progress in Histochemistry and Cytochemistry. 2009;43:177.
[39] Foletti A, Lisi A, Ledda M, de Carlo F, Grimaldi S. Cellular ELF signals as a possible to in informative medicine. Electromag Biol Med. 2009;28:71.
[40] Lisi A, Foletti A, Ledda M, Rosola E, Giuliani L, D'Emilia E, Grimaldi, S. Extremely low frequency 7 Hz 100 uT electromagnetic radiation promotes differentiation in the human peithelial cell line HaCaT. Electromag Biol Med. 2006;25:269.
[41] Ventura C, Maioli M, Asara Y, Santoni D, Mesirca P, Remondini D, Bersani F. Turning on stem cell cardiogenesis with extremely low frequency magnetic fields. FASB J. 2005;19.
[42] Ross S. Combined DC and ELF magnetif fields can alter cell proliferation. Bioelectromagnetics. 2005;11:27.
[43] Tian F, Nakahara T, Yoshida M, Honda N, Hirose H, Miyakoshi J. Exposure to power frequency magnetic fields suppresses X-ray induced apoptosis transiently in Ku80-deficient xrs5 cells. Biochem Biophys Res Commun. 2002;292:355.
[44] Tofani S, Barone D, Cintorino M, De Santi M, Ferrara A, Orlassino R, Ossola P, Peroglio F, Rolfo K, Ronchetto F. Static and elf magnetic fields induce tumor inhibition and apoptosis. Bioelectromagnetics. 2001;22:419.
[45] Santini M, Ferrante A, Rainaldi G, Indovina P, Indovina P. Extremely low frequency (ELF) magnetic fields andapoptosis: a review. Internat J Rad Biol. 2005;81:1.
[46] Takahashi K, Kaneki I, Date M, Fukada E. Effect of pulsing electromagnetic fields on DNA synthesis in mammalian cells in culture. Cellular and Molecular Life Sciences. 1986;42:185.
[47] Litovitz T, Krause D, Montrose C, Mullins J. Temporally incoherent magnetic fields mitigate the response of biological systems to temporally cohreent magnetic fields. bioelectromagnetics. 1994;15:399.
[48] Goodman R, Bassett C, Henderson A. Pulsing electromagnetic fields induce cellular transcription. Science. 1983;220 1283.
[49] Goodman R, Henderson, A. Exposure of salivary gland cells to low-frequency electromagnetic fields alters polypeptide synthesis. Proceed Nat Acad Sci USA. 1988;85:3928.
[50] Sun W, Chiang H, Fu Y, Yu Y, Xie H, Lu D. Exposure to 50 Hz electromagnetic field induces the phosphorylation and activity of stress-activated protein kinase in cultures cells. Electromag Biol Med. 2001;20:415.
[51] Wolf F, Torsello A, Tedesco B, Fasanella S, Boninsegna A, D'Ascenzo M, Grassi C, Azzena G, Cittadini A. 50-Hz extremely low frequency electromagnetic fields enhance cell proliferation and DNA damage; possible involvement of redox mechanism. Biochimica et Biophysica Acta (BBA) Molecular Cell Research. 2005;1743:120.
[52] Regoli F, Gorbi S, Machella N, Tedesco S, Benedetti M, Bocchetti R, Notti A, Gattorini D, Piva F, Principato G. Pro-oxidant effects of extremely low frequency electromagnetic fields in the land snail Helix aspersa. Free Radical biol and med. 2005;39:1620.
[53] Golfert F, Hofer A, Thummler M, Bauer H, Funk R. Extremely low frequency electromagnetic fields and heat shock can increase microvesicle motility in astrocytes. Bioelectromagnetics. 2001;22:71.
[54] Zrimec A, Jerman I, Lahajnar G. Alternating electric fields stimulate ATP synthesis in Escherichia coli Cell Mol Biol Letters. 2002;7:172.
[55] Paksy K, Thuroczy G, Forgacs Z, Lazar P, Gaati I. Influence of sinusoidal 50-Hz magnetic field on cultured human ovarian granulosa cells. Electromag Biol Med. 2000;19:91.
[56] Kula B, Sobczak A, Kuska R. Effects of static and ELF magnetic fields on free-radical processes in rat liver and kidney. Electromag Biol Med. 2000;19:99.
[57] Milani M, Ballerini M, Ferraro L, Zabeo M, Barberis M, Cannone M, Faleri M. Magnetic field effects on human lumphocytes. Electromag Biol Med. 2001;20:81.
[58] Jandova A, Hurych, J, Pokorny, J, Coeek,A , Trojan, S, Nedbalova, M, Dohnalova, A. Effects of sinusoidal magnetic field on adherence inbition of leukocytes. Electromag Biol Med. 2001;20:397.
[59] Weiss P. Cellular Dynamics. Reviews of Modern Physics. 1959;31:11.
[60] Weiss P. Interactions between cells. Reviews of Modern Physics; 1959;31:449.
[61] Bischof M, Del Giudice, E. Communication and the Emergence of Collective Behavior in Living Organisms: A Quantum Approach. Molecular Biology International 2013;Review Article 19 pages.
[62] Tsong T. Electrical modulation of membrane proteins: enforced conformational oscillations and biologial energy and signal transduction. Annu Rev Biophys Biophys Chem. 1990;19:83.
[63] Tsong T. Molecular recognition and processing of periodic signals in cells: study of activation of membrane ATPases by alternatinv electric fields. Biochim Biophys Acta. 1992;1113:53.
[64] Tsong T, Liu DS, Chauvin, F, Astumian, RD. Resonance electroconformational coupling: a proposed mechanism for energy and signal transductions by membrane proteins. Biosci Rep. 1989;9:13.
[65] Beneduci A. Bioelectrochemistry Research Developments. Nova Science Publishers 2008;Ch: Review on the mechanisms of interaction between millimeter waves and biological systems 35.
[66] Foster K, Baish J. Viscous damping of vibration in microtubules J Biol Physics. 2000;26:255.
[67] Karimov A, Shcheglow V. Role of weak energy fluxes in the formation of coherent structures in macromolecular media. J of Russian Laser Res. 2000;21:515.
[68] Berg H. Milestones of bioelectromagnetism: monographs and proceedings. Electromag Biol Med. 2004;23:181.
[69] Sonnier H, Marino A. Sensory transductin as a proposed model for biological detection of electromagnetic fields. Electromag Biol Med. 2001;20:153.
[70] Chiabrera A, Nicolini C, Schwan, H (Eds). Interactions between Electromagnetic Fields and Cells Plenum Press, New York 1985;New York and London.
[71] Challis L. Mechanisms for interaction between rf fields and biological tissue. Bioelectromagnetics. 2005;26:S98.
[72] Ho M-W, Popp F, Warnke U (Eds). Bioelectrodynamics and Biocommunication. World Scientific 1994;New Jersey, London, Hong Kong.
[73] Popp F-A, Warnke U, Konig H, Peschka W (Eds). Electromagnetic Bio-Informaiton. Urban & Schwarzenburg 1989;Munchen, Wien, Baltimore.
[74] Ho M-W. Wireless phone radiation "possibley carcinogenic". Sci in Soc. 2002;51.
[75] Van Wijk R. Bio-photons and bio-communication. J of Sci Explor. 2001;15:183.
[76] Levin M. Bioelectromagnetics in Morphogenesis. Bioelectromagnetics.2003;24:295.
[77] Funk H, Monsees T, Ozkucur N. Electromagnetic effiects - From cell biology to medicine. Progress in histochemistry and cytochemistry. 2009;43:177.
[78] Adey W. Electromagnetics in biology and medicine. In: Matsumoto H, editor 1993;Modern readio science:Oxford University Press.
[79] Levin M. Bioelectric mechanisms in regeneration: unique aspects and furture perspectives. Semin Cell Dev Biol. 2009;20:543.
[80] Levin M. Molecular bioelectricity in developmental biology: New tools and recent discoveries. Bioessays. 2012;34:205.
[81] Cifra M, Fields J, Farhadi A. Electromagnetic cellular interactions. Progress in Biophysics and Molecular Biology. 2011;105:223.
[82] Pilla A. Nonthermal electromagnetic fields: From first messenger to therapeutic applications. Electromagn Biol Med. 2013;32:123.
[83] Burr H, Northrop F. The electrodynamic theory of life. . Quarterly Review of Biology. 1935;10:322.
[84] McLeod K, Rubin CT, Donahue HJ. Electromagnetic fields in bone repair and adaptation. Radio Sci. 1995;30:233.
[85] Otter M, McLeod KJ, Rubin CT. Effects of electromagnetic fields in experimental fracture repair. Clin Orthop. 1998;355:S90.
[86] Bersani F, Marinelli F, Ognibene A, Matteucci A, Cecchi S, Santi S, Squarzoni S, Maraldi NM. Intramembrane protein distribution in cell cultures is affected by 50 Hz pulsed magnetic fields. Bioelectromagnetics. 1997;18:463.
[87] Gordon G. Designed electromagnetic pulsed therapy: clinical applications. J Cell Physiol. 2007;212:579.
[88] Schild L, Plumeyer F, Reiser G. Ca(2+) rise within a narrow window of concentration prevents functional injury of mitochondria exposed to hypoxia/reoxygenation by increasing antioxidative defense. FEBS J. 2005;272:5844.
[89] Ikehara T, Yamaguchi H, Hosokawa K, Houchi H, Park KH, Minakuchi K, Kashimoto H, Kitamura M, Kinouchi Y, Yoshizaki K, Miyamoto H. Effects of a time-varying strong magnetic field on transient increase in Ca2+ release induced by cytosolic Ca2+ in cultured pheochromocytoma cells. Biochim Biophys Acta. 2005;1724:8.
[90] Lieb R, Regelson W, West B, Jordan RL, DePaola, DP. Effect of pulsed high frequency electromagnetic radiation on embryonic mouse tissue palate in vitro. J Dent Res. 1980;59.
[91] McLeod B, Liboff AR, Smith SD. Electromagnetic gating of ion channels. J Theor Biol. 1992;158:15.
[92] Baureus Koch C, Sommarin M, Persson BR, Salford LG, Eberhardt JL. Interaction between weak low frequency magnetic fields and cell membranes. Bioelectromagnetics. 2003;24:395.
[93] Rosen A. Mechanism of action of moderate intensity static magnetic fields on biological systems. Cell Biochem Biophys. 2003;39:163.
[94] Markov M, Colbert AP. Magnetic and electromagnetic field therapy. Journal of Back Musculoskeletal Rehab. 2001;15:17.
[95] Foletti A, Grimaldi S, Lisi A, Ledda M, Liboff AR. Bioelectromagnetic medicine: The role of resonance signaling. Electromagn Biol Med. 2012;32:484.
[96] Bassett A. Biological effects of elctrical and magnetic fields. Academic Press, Inc, 1994;San Diego:13.
[97] Yost M, Liburdy RP. Time-varying and static magnetic fields act in combination to alter calcium signal transduction in the lymphocyte. FEBS Letters. 1992;296:117.
[98] Sun W, Mogadam MK, Sommarin M, Nittby H, Salford LG, Persson BR, Eberhardt JL. Calcium efflux of plasma membrane vesicles exposed to ELF magnetic fields--test of a nuclear magnetic resonance interaction mode. Bioelectromagnetics. 2012;33:535.
[99] Ingber L, Pappalepore M, Stesiak RR. Electroencephalographic field influence on calcium momentum waves. J Theor Biol. 2014;343:138.
[100] Gartzke J, Lange K. Cellular target of weak magnetic fields: ionic conduction along actin filaments of microvilli. Am J Physiol Cell Physiol. 2002;283:C1333.
[101] Selvam R KG, Narayana R, Raju K, Gangadharan A, Manohar B, Puvanakrishnan R. Low frequency and low intensity pulsed electromagnetic field exerts its anti-inflammatory effect through restoration of plasma membrane calcium ATPase activity. Life Sci. 2007;80:2403.
[102] Balcavage W, Alvager T, Swez J, Goff CW, Fox MT, Abdullyava S, King MW. A mechanism of action of extremely low frequency electromagnetic fields on biological systems. Biochem Biophys Res Commun. 1996;222:374.
[103] Ubeda A, Enrique MD, Pascual MA, Parreno A. Hematological changes in rats exposed to weak electromagnetic fields. Life Sci. 1997;61:1651.
[104] Després J. Abdominal Obesity and Cardiovascular Disease: Is Inflammation the Missing Link? Can J Cardiol. 2012;Aug 10. [Epub ahead of print].
[105] Knevel R, van Nies JA, le Cessie S, Huizinga TW, Brouwer E, van der Helm-van, Mil AH. Evaluation of the contribution of cumulative levels of inflammation to the variance in joint destruction in rheumatoid arthritis. Ann Rheum Dis. 2012;Aug 17.
[106] Matarese G, Procaccini C, De Rosa V. At the crossroad of T cells, adipose tissue, and diabetes. Immunol Rev. 2012;249:116.
[107] Westermann D. Does inflammation trigger fibrosis in hypertrophic cardiomyopathy: a burning question? Heart. 2012;98:965.
[108] Zilka N, Kazmerova Z, Jadhav S, Neradil P, Madari A, Obetkova D, Bugos O, Novak M. Who fans the flames of Alzheimer's disease brains? Misfolded tau on the crossroad of neurodegenerative and inflammatory pathways. J Neuroinflammation. 2012;7:47.
[109] Johnson A, Justin Milner J, Makowski L. The inflammation highway: metabolism accelerates inflammatory traffic in obesity. Immunol Rev. 2012;249:218.
[110] Camilleri M, Lasch K, Zhou W. The Confluence of Increased Permeability, Inflammation, and Pain in Irritable Bowel Syndrome. Am J Physiol Gastrointest Liver Physiol. 2012;Jul 26. [Epub ahead of print].
[111] Tufekci K, Meuwissen R, Genc S, Genc K. Inflammation in Parkinson's disease. Adv Protein Chem Struct Biol. 2012;88:69.
[112] Moretti M, Bennett J, Tornatore L, Thotakura AK, Franzoso G. Cancer: NF-?B regulates energy metabolism. Int J Biochem Cell Biol. 2012;Aug 10. [Epub ahead of print].
[113] High K, Brennan-Ing M, Clifford DB, Cohen MH, Currier J, Deeks SG, Deren S, Effros RB, Gebo K, Goronzy JJ, Justice AC, Landay A, Levin J, Miotti PG, Munk RJ, Nass H, Rinaldo CR Jr, Shlipak MG, Tracy R, Valcour V, Vance DE, Walston JD, Volberding P. OAR Working Group on HIV and Aging. HIV and aging: state of knowledge and areas of critical need for research. A report to the NIH Office of AIDS Research by the HIV and Aging Working Group. J Acquir Immune Defic Syndr. 2012;60:S1.
[114] Nielsen C, Bendtzen K. Immunoregulation by naturally occurring and disease-associated autoantibodies : binding to cytokines and their role in regulation of T-cell responses. Adv Exp Med Biol. 2012;750:116.
[115] Nathan C. pints of control in inflammation. Nature. 2002;420:846.
[116] Berger I, Peleg O, Ofek-Shlomai N. Inflammation and early brain injury in term and preterm infants. Isr Med Assoc J. 2012;14:318.
[117] Hovland A, Lappegård KT, Mollnes TE. LDL apheresis and inflammation - implications for atherosclerosis. Scand J Immunol. 2012;Jun 5. doi: 10.1111/j.1365-3083.2012.02734.x. [Epub ahead of print].
[118] Sakurai H. Targeting of TAK1 in inflammatory disorders and cancer. Trends Pharmacol Sci. 2012; Jul 12. [Epub ahead of print].
[119] Zweifach B, Grant L, McCluskey RT. The Inflammatory process. Academic Press 1965;New York.
[120] Weiss S. Tissue destruction by neutrophils. N Engl J Med. 1989;320:365.
[121] Morgan J, Pereira HZ, Sukiennicki T, Spitznagel JK, Larrick JW. Human neutrophil granule cationic protein CAP37 is a specific macrophage chemotaxin that share homology with inflammatory proteinases. Adv Exp Med Biol. 1991;305:89.
[122] Kleemann R, Zadelaar S, Kooistra T. Cardiovasc Res. Cytokines and atherosclerosis: a comprehensive review of studies in mice. 2008;79:360.
[123] Dinarello C. The role of the interleukin-1-receptor antagonist in blocking inflammation mediated by interleukin-1. N Engl J Med. 2000;343:732.
[124] Strober W, James SP. The interleukins. Pediatr Res. 1988;24:549.
[125] Hedrich C, Bream J. Cell type-specific regulation of IL-10 expression in inflammation and disease. Immunol Res. 2010;47:185.
[126] Hehlgans T, Pfeffer K. The intriguing biology of the tumor necrosis factor/tumor necrosis factor receptor superfamily: players, rules and the games. Immunology. 2005;115:1.
[127] Pearson A. Scavenger receptors in innate immunity. Curr Opin Immunol. 1996;8:20.
[128] Janeway C, Medzhitov Jr., R. Innate immune recognition. Annu Rev Immunol. 2002;20:197.
[129] Rosenberger C, Scott MG, Gold MR Hancock RE, Finlay BB. Salmonella typhimurium infection and lipopolysaccharide stimulation induce similar changes in macrophage gene expression. J Immunol. 2000;164:5894.
[130] Kobayashi Y, Miyaji C, Watanabe H, Umezu H, Hasegawa G, Abo T, Arakawa M, Kamata N, Suzuki H, Kodama T, Naito M. Role of macrophage scavenger receptor in endotoxin shock. J Pathol. 2000;192:263.
[131] Rothstein J, Schreiber H. Relationship of tumour necrosis factor and endotoxin to macrophage cytotoxicity, haemorrhagic necrosis and lethal shock. Ciba Found Symp. 1987;131:124.
[132] Gilmore T. Introduction to NF-kB: players, pathways, perspectives. Oncogene. 2006;25:6680.
[133] Sheikh M, Huang Y. Death receptor activation complexes: it takes two to activate TNF receptor 1. Cell Cycle. 2003;2:550.
[134] Ciombor D, Aaron RK, Wang S, Simon B. Modification of osteoarthritis by pulsed electromagnetic field--a morphological study. Osteoarthritis Cartilage. 2003;11:455.
[135] Gómez-Ochoa I, Gómez-Ochoa P, Gómez-Casal F, Cativiela E, Larrad-Mur L. Pulsed electromagnetic fields decrease proinflammatory cytokine secretion (IL-1ß and TNF-a) on human fibroblast-like cell culture. Rheumatol Int. 2011;31:1283.
[136] Benazzo F, Cadossi M, Cavani F, Fini M, Giavaresi G, Setti S, Cadossi R, Giardino R. Cartilage repair with osteochondral autografts in sheep: effect of biophysical stimulation with pulsed electromagnetic fields. J Orthop Res. 2008;26:631.
[137] Vianale G, Reale M, Amerio P, Stefanachi M, Di Luzio S, Muraro R. Extremely low frequency electromagnetic field enhances human keratinocyte cell growth and decreases proinflammatory chemokine production. Br J Dermatol. 2008;158:1189.
[138] Vincenzi F, Targa M, Corciulo C, Gessi S, Merighi S, Setti S, Cadossi R, Borea PA, Varani K. The anti-tumor effect of A3 adenosine receptors is potentiated by pulsed electromagnetic fields in cultured neural cancer cells. PLoS One. 2012;7:e39317.
[139] Ross C, Harrison BS. Effect of pulsed electromagnetic field on inflammatory pathway markers in RAW 264.7 murine macrophages. J Inflamm Res. 2013;6:45.
[140] Natarajan M, Nayak BK, Galindo C, Mathur SP, Roldan FN, Meltz ML. Nuclear translocation and DNA-binding activity of NFKB (NF-kappaB) after exposure of human monocytes to pulsed ultra-wideband electromagnetic fields (1 kV/cm) fails to transactivate kappaB-dependent gene expression. Radiat Res. 2006;165:645.
[141] Selvam R, Ganesan K, Narayana R, Gangadharan A, Manohar B, Puvanakrishnan R. Low frequency and low intensity pulsed electromagnetic field exerts its antiinflammatory effect through restoration of plasma membrane calcium ATPase activity. Life Sci. 2007;80:2403.
[142] Rohde C, Chiang A, Adipoju O, Casper D, Pilla A. Effects of pulsed electromagnetic fields on IL-1b and post operative pain: a double-blind, placebo-controlled pilot study in breast reduction patients. Plast Reconstr Surg. 2009;125:1620.
[143] Yan X, Han J, Zhang Z, Wang J, Cheng Q, Gao K, Ni Y, Wang Y. Lung cancer A549 cells migrate directionally in DC electric fields with polarized and activated EGFRs. Bioelectromagnetics. 2009;30:29.
[144] Delle-Monache S, Angelucci A, Sanità P, Iorio R, Bennato F, Mancini F, Gualtieri G, Colonna RC. Inhibition of angiogenesis mediated by extremely low-frequency magnetic fields (ELF-MFs). PLoS One. 2013;8:e79309.
[145] Haddad J, Obolensky AG, Shinick P. The biologic effects and the therapeutic mechanism of action of electric and electromagnetic field stimulation on bone and cartilage: new findings and a review of earlier work. J Altern Complement Med. 2007;13:485.
[146] Eichwald C, Kaiser F. Model for external influences on cellular signal transduction pathways including cytosolic calcium oscillations. Bioelectromagnetics. 1995;16:75.
[147] Bawin S, Adey W, Sabbott I. Ionic factors in release of 45-Ca2+ from chicken cerebral tissue by electromagnetic fields. Proc Natl Acad Sci USA. 1978;75:6314.
[148] Adey W. The cellular microenvironment and signaling through cell membranes. Prog Clin Biol Res. 1988;257:81.
[149] Aoki H, Yamazaki H, Yoshino T, Akagi T. Effects of static magnetic fields on membrane permeability of a cultured cell line. Res Commun Chem Pathol Pharmacol. 1990;69:103.
[150] Binhi V, Savin A. Effects of weak magnetic fields on biological systems: physical aspects. Physics-Uspekhi. 2003;45:259.
[151] Zimmerman Z, Pennison M, Brezovich I, Nengun Y, Yang C, Ramaker R, Absher D, Myers R, Kuster N, Costa F, Barbault A, Pasche B. Cancer cell proliferation is inhibited by specific modulation frequencies. Br J Cancer. 2012;106:307.
[152] Mills K, McGuirk P, Keogh B, Higgins S, Donnelly G, Leavy O, et al. Regulatory T cells induced by infection: Host immune protection or pathogen immune subversion? Immunol. 2004;113:57.
http://inter-use.com/Journals/JSAB/2015/Volume_03_Issue_02/2015/0302/86.html
Recent ENDV News
- Form 15-12G - Securities registration termination [Section 12(g)] • Edgar (US Regulatory) • 02/02/2024 10:23:02 PM
- Form 8-K - Current report • Edgar (US Regulatory) • 01/03/2024 10:30:14 PM
- Form 10-Q/A - Quarterly report [Sections 13 or 15(d)]: [Amend] • Edgar (US Regulatory) • 12/29/2023 08:20:36 PM
- Form 8-K - Current report • Edgar (US Regulatory) • 12/04/2023 02:08:41 PM
- Form NT 10-Q - Notification of inability to timely file Form 10-Q or 10-QSB • Edgar (US Regulatory) • 11/15/2023 05:21:48 PM
- Form 8-K - Current report • Edgar (US Regulatory) • 10/12/2023 10:03:32 AM
- Form 1-A/A - Offering Statement [Regulation A]: [Amend] • Edgar (US Regulatory) • 08/25/2023 11:04:33 AM
- Form 10-Q - Quarterly report [Sections 13 or 15(d)] • Edgar (US Regulatory) • 08/21/2023 09:16:04 PM
Avant Technologies Equipping AI-Managed Data Center with High Performance Computing Systems • AVAI • May 10, 2024 8:00 AM
VAYK Discloses Strategic Conversation on Potential Acquisition of $4 Million Home Service Business • VAYK • May 9, 2024 9:00 AM
Bantec's Howco Awarded $4.19 Million Dollar U.S. Department of Defense Contract • BANT • May 8, 2024 10:00 AM
Element79 Gold Corp Successfully Closes Maverick Springs Option Agreement • ELEM • May 8, 2024 9:05 AM
Kona Gold Beverages, Inc. Achieves April Revenues Exceeding $586,000 • KGKG • May 8, 2024 8:30 AM
Epazz plans to spin off Galaxy Batteries Inc. • EPAZ • May 8, 2024 7:05 AM